Thyroid Hormone Rhythms and the Brain
Thyroid hormones released in pulses appear to be the pacemaker that ensures all hormone-producing organs give a coordinated response to changing physical, physiological and psychological conditions
[Adapted from my 2006 book, Rhythms of Life, with selected references] 1
As I recently explained, THs are produced in a distinctly pulsatile manner and appear to generate species-specific TH rhythms with distinctive shifts as animals grow and age. Evidence suggests that individual variations in genetically controlled TH rhythms (what I call “TR phenotypes”) must generate coordinated individual variation in morphology, reproduction and behavior within populations.
Selection for any manifestation of a particular TR phenotype in an ancestral population selects all traits under thyroid hormone control, resulting in rapid and well-coordinated changes in descendants.
With regard to human evolution, the concept provides the first really plausible explanation for a number of phenomena, including the convergent evolution of bipedalism in early hominids, species-specific sexual dimorphism, coordinated changes in morphology, brain function and gut length over time in hominids, cold adaptation in Homo neanderthalensis, the possible independent evolution of H. sapiens in Asia, and regional adaptation of hominid populations.
This new paradigm also provides a unique theoretical framework for explaining recurring human health issues.
But first, some details about these hormone rhythms that should help you understand how the concept works and a page you might want to come back to later for reference.
The human thyroid hormonal cascade from signals originating in the brain to release of hormones from the thyroid gland and actions of thyroid hormones on target tissues in the body — as well as their individual feedback mechanisms — are usually described in rather simplistic terms, as shown in the image below:
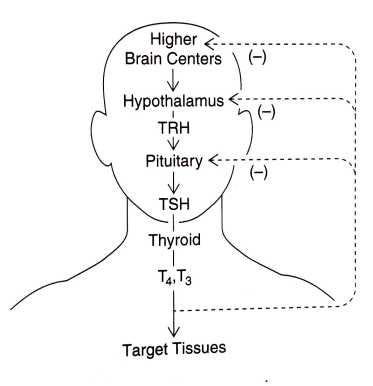
A similar but more detailed model explains the release of all critical hormones, as I show below.
In particular, the pineal gland in the brain receives intermittent electrical signals via the retina and other neural receptors, and releases pulses of melatonin to stimulate the hypothalamus gland (also located in the brain) to release a number of activating hormones, one of which is called growth-hormone releasing hormone.2
The hypothalamus is special in that it responds to melatonin but can also be directly stimulated by electrical signals from other parts of the brain.
Both of these glands translate electrical input messages into chemical output messages, which is what the rest of the body is waiting to hear.
The pituitary gland is the grand intermediary in this chemical messaging scheme: the pituitary is capable of producing a number of hormones in response to the pulsating hormonal signals from the hypothalamus. These pituitary hormones relay the signals out to other hormone-producing organs, including the thyroid gland, the adrenal gland, and the gonads, as shown below.
In this way, pulsating electrical signals received by the two neurohormone-producing organs in the brain (the pineal and the hypothalamus) cause all hormones stimulated by the pituitary, including thyroid hormone, to be secreted into the bloodstream in a distinctly rhythmic manner.
The pulsating nature of hormone release is probably not accidental: indeed, it appears that rhythmic production may be essential to the proper activity of all hormones. For example, it’s been found that continued exposure of tissues to the neurohormone melatonin soon makes them unresponsive, so that increasing levels of it eventually have no effect at all.
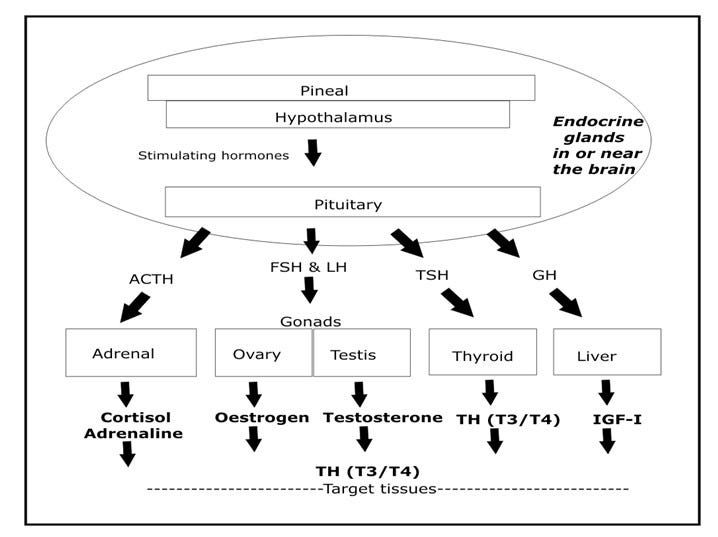
However, in reality, the production and actions of these hormones is much more complex, as the model shown below shows (where flow starts from the bottom right) because of the known need for thyroid hormone to be present at many stages in order for other hormones to be released or to act as they should.
Note that this cascade model I’ve put together does not show the direct nerve connection between the hypothalamus and the thyroid gland (discussed in more detail below), which bypasses the pituitary and allows immediate release of TH in response to direct nervous system stimulation.3
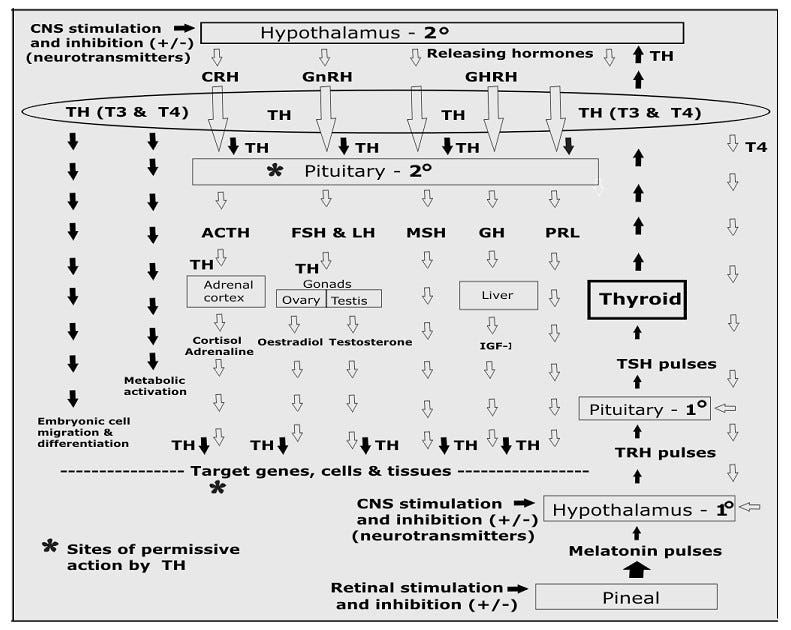
The bottom line is this: TH release must be the first response to any stimulus, because TH must be present at the pituitary for the release of many other hormones (including sex hormones), and again at the site of target tissue, for other hormones to have appropriate effects. These are known as “permissive” actions.4
It works like this: Pulsatile release of pineal melatonin stimulates pulsatile secretion of thyrotropin-releasing hormone (TRH) from the hypothalamus.5 TRH pulses then stimulate bursts of TSH from the pituitary, and TSH pulses finally stimulate pulsatile release of THs from the thyroid glands.
However, in addition, the thyroid gland is now known to have a direct nerve connection (via sympathetic nerves) to the suprachiasmatic nucleus (SCN) of the hypothalamus. [see footnote 3]
This means that thyroid hormone secretion can be stimulated or depressed independently of changing TSH levels, and this immediacy is critical for the ability of organisms to response to rapidly changing environmental conditions.6
Genetic control over this pulsatile hormonal cascade seems to come from eight or more “clock genes” that reside in circadian oscillator cells of the mammalian SCN.
The interaction of these genes with each other to generate a neurohormonal or an electrical output appears to control basic circadian timing. Because as yet unknown “clock-modulating” genes are thought to exist as well, the mechanism is far from being well understood.7
The SCN appears to be developed and functional in a number of laboratory animals by the late fetal stage or, at the very latest, by the early postnatal period. However, it cannot be emphasized too strongly that although circadian rhythms do modulate hormonal rhythms, the two are not identical; thyroid rhythms are known to shift during development in amphibians even when light/dark cycles are constant.8
Thus, it remains to be seen precisely which “clock genes” affect thyroid rhythms and which affect circadian rhythms specifically. In addition, it is also apparent that the genetic basis for differences in overall thyroid hormone metabolism doesn’t lie exclusively in the genes that govern the secretion of thyroid hormone.
Variation is known or suspected to exist in the conformation or concentration of independent genes that produce proteins controlling thyroid hormone distribution in the blood and cerebral fluid (e.g., transthyretin), or that encode thyroid hormone receptors, receptor ligands and/or cofactors in target tissues.9
However, in all animals, the precise pattern of thyroid rhythms are known to change both seasonally and daily according to other physiological demands. Fluctuations in thyroid rhythms also occur with age, reproductive stage, psychological state and general health.
As amounts of thyroid hormone released are known to vary relative to the many factors described above, static measurements (single samples) of blood thyroid hormone concentrations (or of its pituitary harbinger, thyroid stimulating hormone, TSH), which are often used to characterize thyroid hormone function, can’t actually be reliably compared between species or even between individuals.10
In part, this is because any two samples drawn are unlikely to have been taken under identical conditions.
However, a few tests exist that may serve as proxies for the existence of thyroid rhythms because they document differences in thyroid hormone metabolism between species. One such test measures the time taken for a measured amount of thyroid hormone (in its T4 form) to become reduced to half its original value (called its half-life), a test that reflects differences in thyroid hormone metabolism after thyroid hormone is released.
Here we see that the average half-life of T4 is 13 hours in dogs, 16.6 hours in cats, and 6.8 days in humans—in other words, carnivores like dogs and cats can (or must) replenish T4 levels much more often than omnivorous humans (I’ll discuss such differences in thyroid hormone metabolism between carnivores, herbivores and omnivores in a future post).11
Some differences between animal breeds have also been demonstrated. For example, the half-life of T4 in the beagle (a dog that typically breeds twice a year) is twice as high as that found in the basenji (a dog that typically breeds only once a year).
So although comparisons within and between species for measured values of thyroid hormone are problematic, it’s nevertheless apparent that significant species-specific differences in thyroid hormone metabolism exist.
A few studies have actually sampled thyroid hormone levels frequently enough to document the normal daily fluctuating pattern of thyroid hormone secretion in several species. These studies suggest not only that marked differences do exist between species for daily rhythms of thyroid hormone secretion but that individual differences in daily rhythms within species also exist.12
In summary, I propose that populations adapt to changing environmental conditions over geological time using the same hormonal mechanisms that individuals use to adapt to daily and seasonal changes.
This concept offers the first really plausible explanation for how sexual dimorphism, polyphyletic origins and convergent evolution are achieved, and thus provides an especially useful framework for examining hominid evolution and the health implications of our unique thyroid metabolism history.
Crockford, S.J. (2003). Thyroid rhythm phenotypes and hominid evolution: a new paradigm implicates pulsatile hormone secretion in speciation and adaptation changes. Comparative Biochemistry and Physiology Part A 135, 105–129.
Crockford, S.J. (2006). Rhythms of Life: Thyroid Hormone and the Origin of Species. Trafford, Victoria.
Korf, H-W. (1994). The pineal organ as a component of the biological clock: phylogenetic and ontogenetic considerations. The Aging Clock: The Pineal Gland and Other Pacemakers in the Progression of Aging and Carcinogenesis, W. Pierpaoli et al. (eds.), Annals of the New York Academy of Science 719, New York, p. 13-42.
Kalsbeek, A., Fliers, E., Franke, A.N., Wortel, J. and Buijs, R.M. (2005). Functional connections between the suprachiasmatic nucleus and the thyroid gland as revealed by lesioning and viral tracing techniques in the rat. Endocrinology 141(10), 3832-3841.
Hadley, M.E. (2000). Endocrinology, Fifth Edition. Prentice-Hall Inc, New Jersey.
Hulbert, A.J. (2000). Thyroid hormones and their effects: A new perspective. Biological Review 75, 519–631. https://doi.org/10.1017/S146479310000556X
Haisenleder, D.J., Ortolano, G.A., Dalkin, A.C., et al. (1992). Differential actions of thyrotropin (TSH)-releasing hormone pulses in the expression of prolactin and TSH subunit messenger ribonucleic acid in rat pituitary genes in vitro. Endocrinology 130, 2917-2923.
Wright, M. L. (2002). Melatonin, diel rhythms, and metamorphosis in anuran amphibians. General and Comparative Endocrinology 126, 251-254.
Zwahlen, J., Gairin, E., Vianello, S., et al. 2024 The ecological function of thyroid hormones. Philosophical Transactions of the Royal Society B, 379, 20220511. https://doi.org/10.1098/rstb.2022.0511
Reppert, S.M. and Schwartz, W.J. (1986). Maternal suprachiasmatic nuclei are necessary for maternal coordination of the developing circadian system. Journal of Neuroscience 6, 2724-2729.
Reppert, S.M. and Weaver, D.R. (2002). Coordination of circadian timing in mammals. Nature 418, 935-941.
Wright, M. L. (2002). Melatonin, diel rhythms, and metamorphosis in anuran amphibians. General and Comparative Endocrinology 126, 251-254.
See additional references in Crockford, S.J. (2006). Rhythms of Life: Thyroid Hormone and the Origin of Species. Trafford, Victoria.
Anderson, G.W., Mariash, C.N. and Oppenheimer, J.H. (2000). Molecular actions of thyroid hormone. Werner and Ingbar’s The Thyroid, Eighth Edition, L.D. Braverman and R.D. Utiger (eds.), Lippincott, Philadelphia, p. 174-195.
Huang, H., Cai, L., Remo, B.F. and Brown, D.D. (2001). Timing of metamorphosis and the onset of the negative feedback loop between the thyroid gland and the pituitary is controlled by type II iodothyronine deiodinase in Xenopus laevis. Proceedings of the National Academy of Sciences USA 98(13), 7348-7353.
Wrutniak, C., Casa, F. and Cabello, G. (2001). Thyroid hormone action in mitochondria. Journal of Molecular Endocrinology 26, 67-77.
Gagneux, P., Amess, B. Diaz, S., Moore, S., Patel, T., et al. (2001). Proteomic comparison of human and great ape blood plasma reveals conserved glycosylation and differences in thyroid hormone metabolism. American Journal of Physical Anthropology 115, 99-109.
Kaptein, E.M., Hays, M.T. and Ferguson, D.C. (1994). Thyroid hormone metabolism: a comparative evaluation. Thyroid disorders, Veterinary Clinics of North America, Small Animal Practice 24, D.C. Ferguson (ed.), W.B. Saunders Co., Philadelphia, p. 431-463.
Gancedo, B., Alonso-Gomez, A.L., de Pedro, N., et al. (1996). Daily changes in thyroid activity in the frog Rana perizi: Variations with season. Comparative Biochemistry and Physiology C. 114, 79–87. https://doi.org/10.1016/0742-8413(96)00009-6
Gancedo, B., Alonso-Gomez, A.L., de Pedro, N., et al. (1997). Changes in thyroid hormone concentrations and total contents through ontogeny in three anuran species: evidence for daily cycles. General and Comparative Endocrinology 107, 240–250. https://doi.org/10.1006/gcen.1997.6922
Wright, M.L., Duffy, J.L., Guertin, C.J., et al. 2003. Developmental and diel changes in plasma thyroxine and plasma and ocular melatonin in the larval and juvenile bullfrog, Rana catesbeiana. General and Comparative Endocrinology 130, 120-128.